NML Review | Professor Fan Zhuangjun: Research Progress of Transition Metal Compound-Carbon Hybrid Electrode for High Energy/High Power Density Supercapacitors
In order to increase the energy density of supercapacitors, transition metal compounds (TMC) with higher theoretical specific capacitance are usually used as electrode active materials. However, due to problems such as low intrinsic conductivity and large volume expansion during charging and discharging, the power density and cycle life are low, which severely hinders large-scale applications. Recently, the composite of TMC and conductive carbon skeleton is considered to be an effective solution to the above-mentioned challenges. Here, this article will review the latest developments in TMC/carbon hybrid electrodes with high energy/power density in terms of structural design strategies.
Highlights of this article
1. The research progress of transition metal compound-carbon hybrid electrodes for high energy/power density supercapacitors is reviewed.
2. Discuss the influence of conductive carbon framework, interface engineering and electronic structure on transition metal compound-carbon hybrid electrode.
3. Put forward some opinions and suggestions on the development of supercapacitors in the future.
brief introduction
In this article, Professor Fan Zhuangjun’s research group from China University of Petroleum (East China) first briefly introduced the energy storage mechanism of supercapacitors, and then systematically reviewed the latest developments in the field of transition metal compound/carbon composite electrodes in the field of supercapacitor electrodes, and further discussed the conductive carbon skeleton The influence of the construction of the transition metal compound/carbon interface engineering and the electronic structure regulation of the transition metal compound on the electrochemical performance. Finally, the challenges and development prospects faced by the development of this type of composite materials, as well as possible future development strategies and research directions are proposed.
Graphic guide
I Conductive carbon skeleton
Due to poor inherent conductivity and slow oxidation-reduction reaction kinetics, the high power density of TMC-based electrodes is usually limited. In order to solve these problems, carbon framework composite materials are usually used. The carbon framework can not only construct effective electron/ion transfer channels, but also maintain the structural stability of the entire electrode. In recent years, carbon skeletons of various sizes (0, 1, 2, 3D) have been successfully designed and introduced into TMC composite materials.
Figure 1. Comparison of physical and chemical properties of different sizes of carbon.
1.1 0D carbon (carbon or graphene quantum dots)
0D carbon materials, such as carbon quantum dots (CQDs) or graphene quantum dots (GQDs), have high electron mobility and large specific surface area due to their small size effect, quantum tunneling effect, Coulomb blocking effect, surface effect, etc. The characteristics have been extensively studied in supercapacitors. Under this circumstance, the combination of TMC and OD carbon materials has become a potential supercapacitor electrode material. For example, Ji et al. successfully embedded reduced carbon quantum dots (RCQDs) into RuO₂ nanoparticles (RCQDs/RuO₂) through a simple impregnation method (Figure 2a). The hybrid network constructed by RCQDs/RuO₂ can provide fast electron transport and ion migration paths. In addition, the introduction of the overall structure of RCQDs can effectively prevent the further agglomeration of RuO₂ nanoparticles and greatly promote the rapid ion/electron transfer during charge and discharge. In addition, GQDs can be uniformly introduced to the surface of MnO₂ nanosheets through hydrothermal and plasma-enhanced chemical vapor deposition processes to obtain a series of GQDs/MnO₂ composite materials. Thanks to the high conductivity and abundant active sites of GQDs (Figure 2b), the maximum specific capacitance of GQDs/MnO₂-3 (3 is the mass ratio of MnO₂/GQDs) at 5 mV s⁻1 can reach 1170 F g ⁻1, has good cycle performance (retention rate is 92.7% after 10,000 cycles).
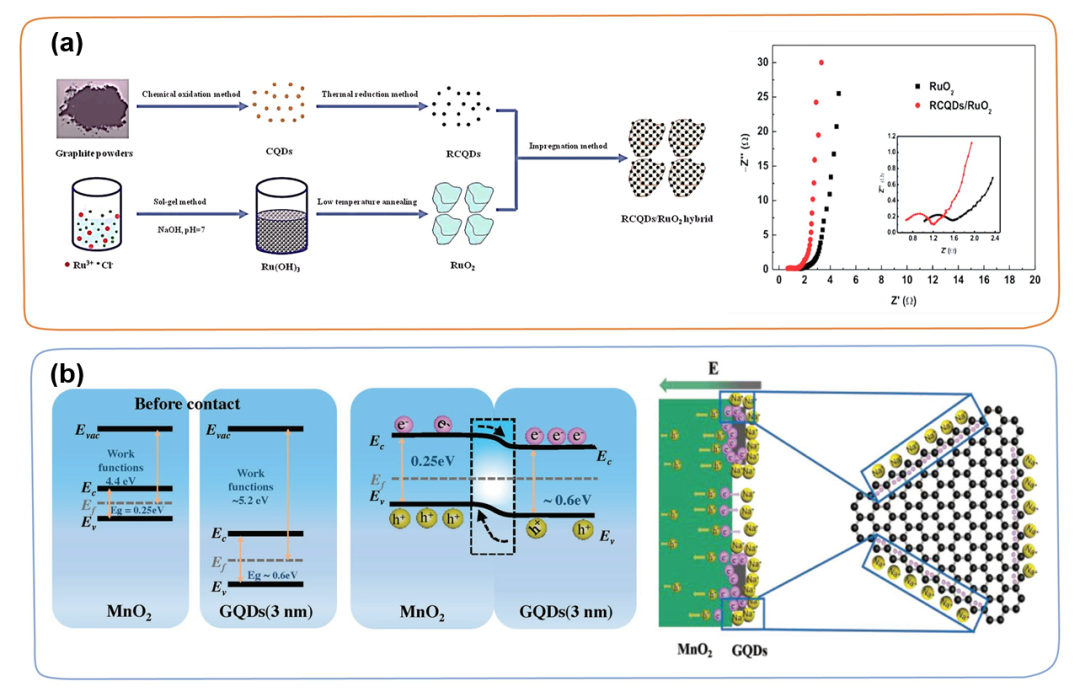
Figure 2. (a) Schematic diagram of the preparation process of RCQDs/RuO₂ hybrid and the comparison of RuO₂ and RCQDs/RuO₂ Nyquist impedance diagrams. (b) Energy map of MnO₂ and GQDs (≈3 nm) before contact and after formation of heterojunction, as well as a schematic diagram of free electrons gathering near the surface of GQDs.
1.2 1D carbon (nanotube or nanofiber)
Due to the high conductivity, good flexibility and superior functionality of one-dimensional carbon materials such as carbon nanofibers (CNF) and carbon nanotubes (CNTs), they have been widely used as single electrode materials or conductive substrates for supercapacitors. In this process, TMC is usually introduced to the surface of 1D carbon in situ by physical or chemical methods. This strategy can not only effectively control the morphology of TMC, but also greatly improve the overall conductivity of the one-dimensional TMC/carbon composite material, which is beneficial to the improvement of power density and energy density. For example, Li et al. used dicyandiamide (DCDA) and polyaniline (PANI) as CNF precursors, and nickel acetylacetonate as a source of Ni, and prepared NiO nanoparticles composite N-doped porous CNF (NiO/PCNF) by electrospinning. Membrane electrode. NiO nanoparticles are uniformly dispersed in the N-doped porous CNF framework (Figure 3). This one-dimensional fiber feature and intertwined conductive skeleton facilitate the rapid transfer of electrolyte ions/electrons in the plane. The maximum specific capacitance of NiO/PCNF-0.75 (0.75 represents the weight ratio of DCDA to nickel acetylacetone) at 1 A g⁻1 is 850 F g⁻1, and it remains 748 F g⁻1 even at 10 A g⁻1 High specific capacitance, which means excellent rate capability. In addition, due to its high conductivity, excellent mechanical strength and flexibility, one-dimensional TMC/carbon composite materials have also been widely studied in the field of wearable device applications.
Figure 3. (a) Schematic diagram of the synthesis of an independent NiO/PCNF composite membrane. (b-d) Scanning electron microscope, CV curve and GCD curve of NiO/PCNF-0.75.
1.3 2D carbon
Due to the ultra-high specific surface area (2630 m2 g⁻1) and excellent electrical conductivity, since the first report by Novoselov and Geim in 2004, the application of 2D graphene-based materials for energy storage and conversion research has rapidly risen. More specifically, graphene as a conductive substrate and flexible matrix has also been extensively studied in the synthesis of graphene/TMC composites. The continuous conjugated sp2-C framework of graphene can provide efficient ion/electron transfer channels, which is not only conducive to improving the rate performance of TMC, but also has important significance for the stability of long-term charge and discharge cycles. For example, Fan et al. prepared Ni(OH)₂/graphene hybrid materials by microwave heating. Structural characterization showed that the prepared Ni(OH)₂ has a hierarchical, flower-like morphology, and was uniformly modified on the graphene nanosheets. Compared with pure Ni(OH)₂, hybrid materials have higher specific capacitance and higher rate performance (as shown in Figure 4a). In addition, Jiang et al. developed a simple, efficient and economical one-pot ball milling method to produce a series of graphene/Co₃O₄ composite materials. The SEM image shows that the nano-Co₃O₄ is uniformly fixed on the graphene surface. Graphene as a conductive skeleton can provide a fast ion transport path. Compared with other composite materials, the prepared graphene/Co₃O₄-2 (2 represents graphene content) shows the most significant specific capacitance of 530 F g⁻1 at 20 A g⁻1 (Figure 4b). When an asymmetric supercapacitor is assembled with graphene as the anode and graphene/Co₃O₄-2 as the cathode, the energy density can reach 67.5 Wh kg⁻1 at a high power density of 0.8 kW kg⁻1.
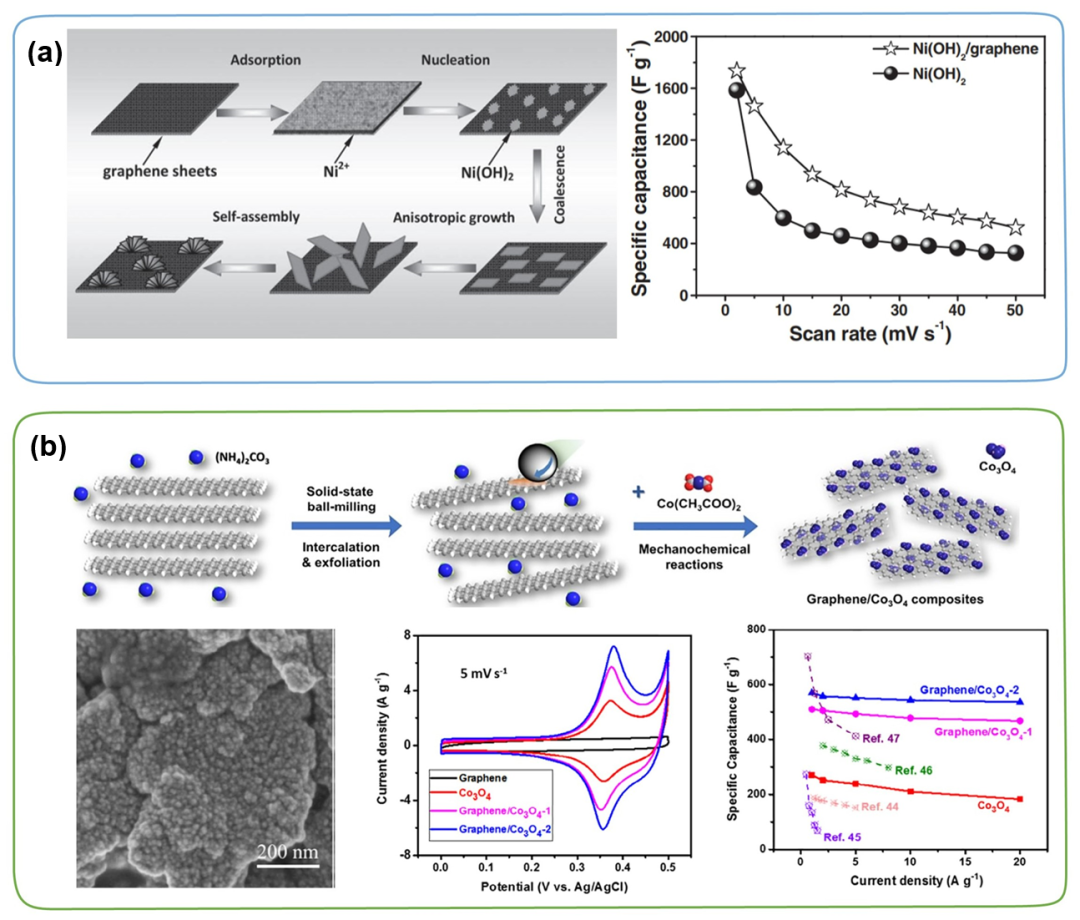
Figure 4. (a) Schematic diagram of the preparation of Ni(OH)₂/graphene, and the specific capacitance of Ni(OH)₂/graphene and Ni(OH)₂ at different scan rates. (b) Schematic diagram of graphene/Co₃O₄ composite preparation, SEM image of graphene/Co₃O₄-2, CV curve of graphene, Co₃O₄, graphene/Co₃O₄-1 and graphene/Co₃O₄-2 at 5 mV s⁻1 , And the specific capacitance at different current densities.
1.4 3D carbon
In order to solve the above-mentioned aggregation problem of graphene, people have proposed a reasonable construction of a three-dimensional carbon skeleton of a highly conductive substrate, such as three-dimensional porous carbon, three-dimensional graphene building, and three-dimensional cross-linked carbon mesh. These carbon frameworks with a unique three-dimensional morphology usually have a hierarchical porous structure and an ultra-high specific surface area, which provides abundant electrolyte ion/electron transfer channels and sufficient growth space for TMC. Bao et al. prepared a three-dimensional graphene framework/Co₃O₄ composite material by thermal explosion method. As shown in Figure 5a, a three-dimensional graphene framework with large pores as a conductive framework can promote the rapid transfer of electrolyte ions/electrons. In addition, Co₃O₄ nanoparticles with a diameter of about 4.6 to 9.4 nm are uniformly and densely distributed on the three-dimensional carbon framework. When the 3D graphene/Co₃O₄ composite material is used as the electrode material of the supercapacitor, when the current density is 1 A g⁻1, the specific capacitance is ≈1765 F g⁻1, and the current density is 10 A g⁻1 under high constant current conditions After 5000 cycles, the specific capacitance remains 93%. In addition, VN nanoparticles combined with 3D carbon matrix (3D VN/C) hybrid materials (Figure 5b) and MOFs derived ZnCoNiS nanoparticles based on 3D rGO/CNTs conductive substrate (CoZnNiS/CNTs/rGO) The sheet array (Figure 5c) has also been studied, and it has been confirmed that the introduction of a three-dimensional carbon network makes the entire electrode have higher conductivity, greatly promotes the electron transfer kinetics, and shortens the diffusion path of electrolyte ions, thereby achieving a combination of Excellent electrochemical performance with high energy density and high power density.
Figure 5. (a) SEM image of the three-dimensional graphene framework/Co₃O₄ composite material and the CV curve of each sample under 50 mV s⁻1. (b) VN/C manufacturing strategy schematic diagram and Ragone diagram. (c) The preparation of CoZnNiS/CNTs/rGO composite material and the schematic diagram of its volume and mass capacitance under different current densities.
In summary, whether it is 0D, 1D, 2D or 3D carbon framework, it is very important to design a carbon structure with high conductivity and large specific surface area to meet the high-quality load of TMC. In addition, how to design an ideal carbon skeleton with both high bulk density and porous structure seems to be a huge challenge. In particular, the relationship between the energy density of the overall device and the ratio of TMC to carbon-based host has not been reported yet. The content of the porous carbon body affects the conductivity and capacitance of the entire electrode. Too high a content of the porous carbon body will achieve a high volume density and result in a higher electrolyte consumption, and a too low content will result in a high charge resistance. In the practical application of TMC, the rapid reaction kinetics of the pseudocapacitor energy storage process generally requires the synergistic effects of electrical conductivity, ion transfer channels, and interface stability. Therefore, the system design of the carbon skeleton in the TMC/carbon-based supercapacitor with high energy/power density may be a future research direction.
II Interface Engineering
In addition to the construction of the conductive carbon skeleton, the well-designed interface engineering between the carbon skeleton and TMC is another indispensable function of the TMC-based electrode, which can significantly increase the energy density and cycle life. Due to the nano-size effect, TMC nanoparticles with high surface energy tend to accumulate during energy storage, which directly leads to capacity degradation and hinders the large-scale application of TMC/carbon-based supercapacitors. In addition, the simple physical combination of TMC and the carbon skeleton usually leads to high interfacial impedance, cutting off the electron transfer channel on the non-uniform interface. Therefore, the ideal carbon/TMC interface design is very important to further improve the electrochemical performance.
2.1 Heteroatom-doped carbon skeleton
Doping the carbon skeleton with heteroatoms (such as B, N, P, S, etc.) is an effective way to build a strong TMC-carbon interface. DFT calculations verified that the density of states and charge distribution of the carbon materials have changed significantly after heteroatom doping. Previous studies have shown that heteroatom doping significantly improves the conductivity and wettability of carbon materials, which is beneficial to improve the electrochemical activity of TMC/carbon-based electrodes. For example, Dubal and Abdel-Azeim et al. proved that the introduction of heteroatoms in the carbon matrix can increase the binding energy between TMC and carbon. In this case, Yang et al. successfully used urea as a nitrogen source and inserted ultrafine MnO nanoparticles into nitrogen-rich carbon nanosheets (MnO@NCs) through a simple strategy (Figure 6a-c). As shown in Figure 6b, the successful introduction of N atoms into the carbon framework facilitates the uniform fixation of ultra-small MnO nanoparticles (about 2-4 nm) on the N-rich carbon nanosheets. In addition, the abundant nitrogen doping in the carbon skeleton changes the local electron distribution, and the nanoparticles preferentially nucleate in situ on the nitrogen-rich sites, which is conducive to the uniform dispersion of the nano-MnO particles and provides for the large-mass loading of the nano-MnO particles. Strong support. Hou et al. synthesized Ni/Co-OOH nanosheets modified with N, S co-doped carbon fragments (NSCF) by in-situ electrochemical self-reconstruction method (Figure 6d-f). Even under high-quality loads, Ni/Co-OOH/NSCF electrodes still provide ultra-high capacity and excellent rate capability
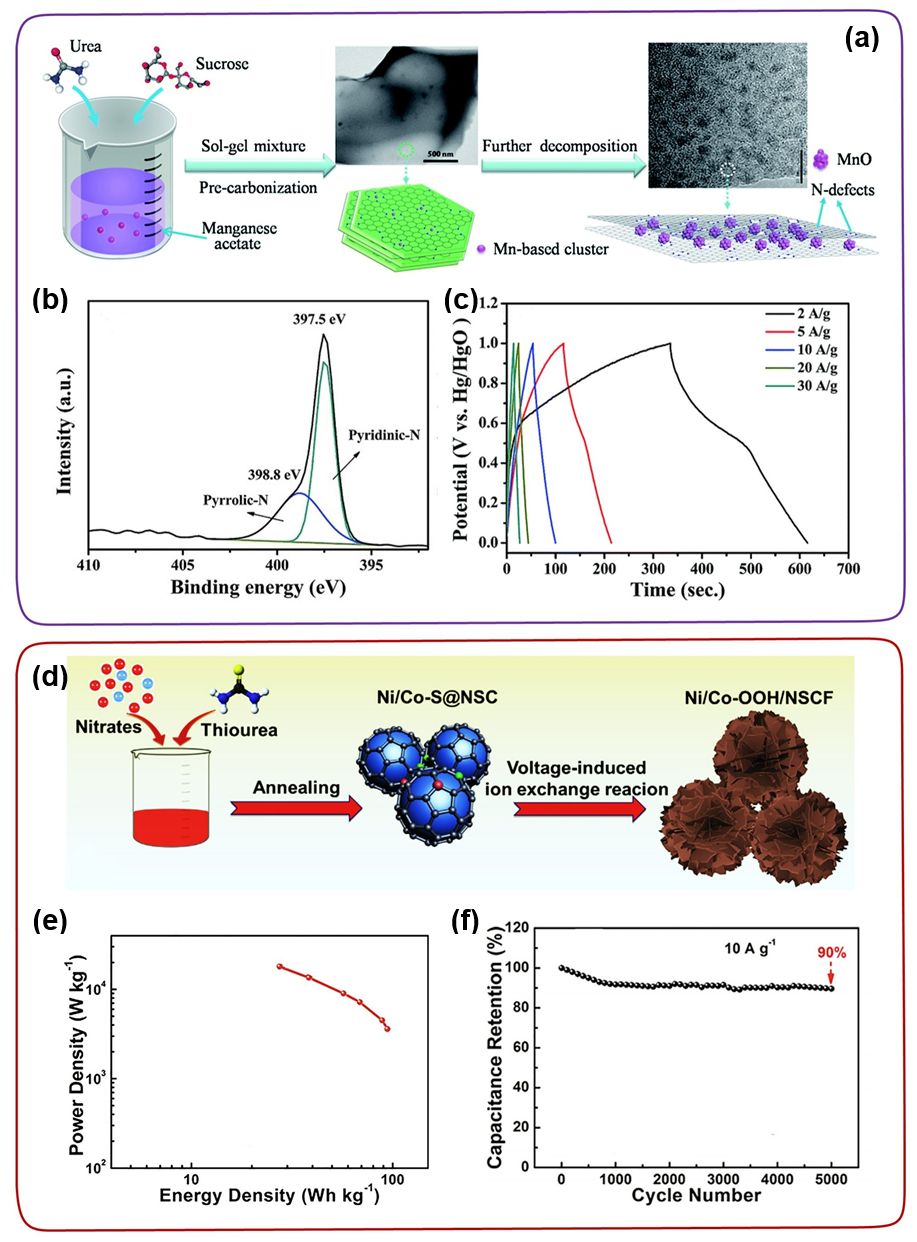
Figure 6. (a) Schematic diagram of the synthesis of MnO@NCs. (b) High-resolution XPS of the N 1s peak of MnO@NCs. (c) Constant current charge and discharge curve of MnO@NCs. (d-f) Synthesis diagram, Ragone diagram and cycle performance diagram of Ni/Co-OOH/NSCF.
2.2 Covalent heterogeneous interface
Similar to heteroatom doping, the introduction of functional groups on the surface of the carbon skeleton is another necessary strategy. By constructing MO/N/P/SC bonds, it is ensured that TMC can form an effective covalent graft with the carbon skeleton, where M represents a transition metal , O/N/P/S stands for functional group, C stands for carbon skeleton. The use of strong oxidizing acids (such as nitric acid and sulfuric acid) to functionalize the carbon surface is the most common method. It can introduce functional groups such as amino, carboxyl, hydroxyl, phosphate, or thiol on the surface of carbon materials. These surface functional groups can not only control the loading of TMC, but also increase the penetration of electrode materials in the electrolyte. More importantly, the covalent interface between TMC and carbon helps to improve the stability of the structure during long-term charging and discharging. For example, Jia et al. wrapped the multi-shell modified Mn₃O₄ hollow spheres on the surface of reduced graphene oxide (rGO), and obtained the Mn₃O₄-rGO hybrid material through the oxygen vacancy-assisted hydroxyl modification method (Figure 7a). Reduced graphene oxide and multi-shell Mn₃O₄ hollow spheres can form Mn-C-O bonds with strong binding force. The XPS O1s spectrum shows the presence of Mn-O-C ratio peaks. As shown in Figure 7b-d, Mn₃O₄-rGO-2 (2 represents different rGO weight) with a larger Mn-OC content (42.1%) has a higher specific capacitance (561.5 C g⁻ at 1 A g⁻1) 1) and better cycle stability (98% retention after 10,000 cycles). Compared with the other two hybrid materials, the improvement of its electrochemical performance is attributed to the construction of a covalent heterogeneous interface, which can ensure rapid charge-discharge reaction kinetics and ultra-long cycle stability.
Figure 7. (a) Schematic diagram of the preparation of Mn₃O₄-reduced graphene oxide. (b) O 1s nuclear energy level spectrum of Mn₃O₄-rGO. (c) The specific capacitance of each sample at different current densities. (d) The content of Mn-O-C and Mn-O-Mn in all Mn₃O₄-rGO samples.
2.3 Organic transition layer
In order to improve the stability of the interface between TMC and the carbon framework, it is also an effective method to covalently introduce an organic transition layer into the carbon surface to form a ternary composite material. The organic transition layer between the carbon skeleton and TMC can form a strong adhesive layer like glue, connecting the conductive carbon skeleton with the TMC with pseudocapacitance. In recent years, conductive polymers such as polyaniline (PANI), polypyrrole (PPy), and polythiophene (PTh) have been extensively studied as carbon coatings. For example, Zhu et al. prepared CuO-PANI-rGO ternary hybrids by hydrothermal treatment after in-situ polymerization (Figure 8). The introduction of polyaniline can not only maintain the strong adsorption between CuO nanorods and the surface of reduced graphene oxide, but also build a rapid charge transfer transport channel between CuO and reduced graphene oxide. The results show that the CuO-PANI-rGO ternary hybrid device has a specific capacitance of 634.4 F g⁻1 and 1 A g⁻1 in an aqueous solution, and achieves 126.8 Wh kg⁻1 at a power density of 114.2 kW kg⁻1. High energy density.
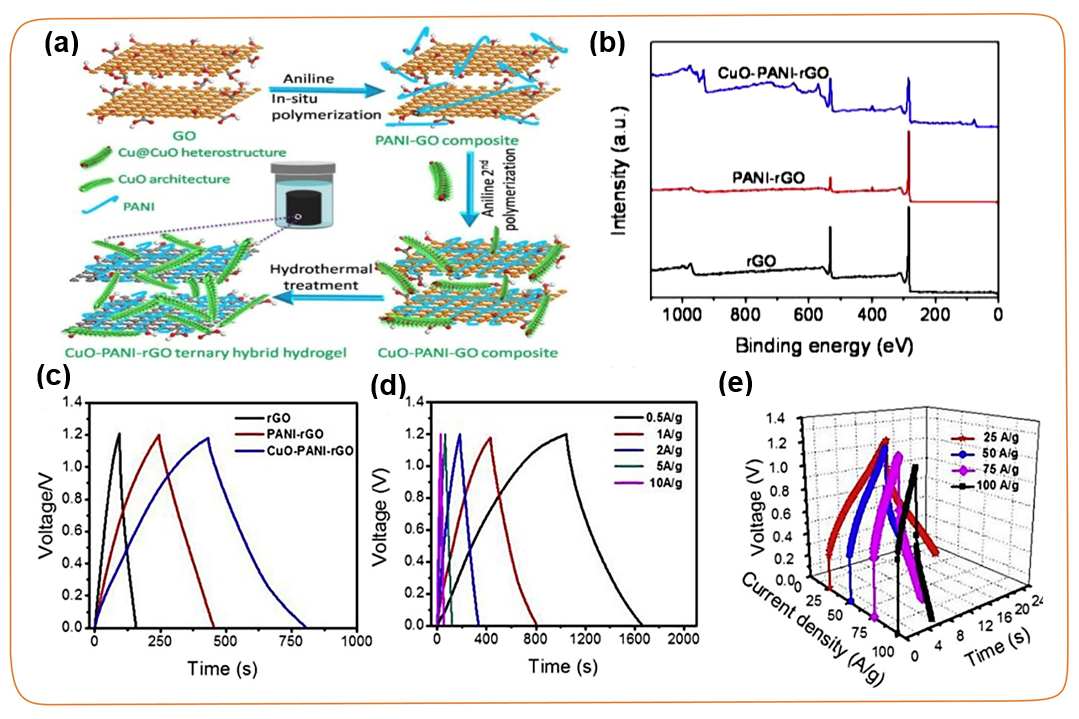
Figure 8. (a) Schematic diagram of the self-assembly process of CuO-PANI-rGO ternary mixed sample. (b) XPS spectra of pure reduced graphite oxide, PANI-rGO binary hybrid, and CuO-PANI-rGO ternary hybrid samples. (c) GCD comparison curve of CuO-PANI-rGO ternary mixed sample and rGO and PANI-rGO samples at a current density of 1.0 A g⁻1. (d-e) GCD curves of CuO-PANI-rGO ternary mixed samples at 0.5~100.0 A g⁻1 different current densities.
2.4 Space constraints
In addition, in order to construct an efficient bonding interface between the carbon skeleton and TMC, the in-situ spatial confinement effect is also an effective strategy for the preparation of TMC/carbon composites. Due to the physical interaction between the carbon surface and the nanocrystals, the nanocrystals are strongly confined in the pores or interlayers of the carbon skeleton. The space confinement effect can not only prevent the carbon nanotubes from leaving the carbon surface directly during the long-term charging and discharging process, but also facilitate the construction of rapid redox reaction kinetics based on electron transfer channels. Because the carbon skeleton has good electrical conductivity and mechanical strength, the charge transfer performance of the TMC/carbon composite material has been greatly improved, and the structural stability has been correspondingly maintained. This is useful for improving the power/energy density and length of the material Cycle life has great advantages. For example, Cao et al. prepared a porous reduced graphene oxide/MoO₃ composite material, through simple mixing of Mo-MOFs and reduced graphene oxide flakes, and then through an annealing process, the reduced graphene oxide nano flakes strongly envelop the MoO₃ particles. As shown in Figure 9a, compared with the original MoO₃, the rate performance and cycle stability of the rGO/MoO₃ composite have been greatly improved. This is mainly due to the rGO network providing a highly conductive and porous channel, allowing electrons The rapid transmission of and ions greatly reduces the impedance, which is beneficial to improve the rate performance. In addition, the reduced graphene oxide film coated with MoO₃ prevents the separation and aggregation of MoO₃ nanomaterials, which greatly improves the cycle performance of MoO₃ nanomaterials. Similarly, Li et al. prepared carbon nanosheets/VN nanodots (VNNDs/CNSs) hybrid electrode materials through a simple space restriction strategy, and inserted VN nanodots (VNNDs) into carbon nanosheets (CNSs) (Figure 9b) ). This strategy can make VNNDs well dispersed in the columnar layered structure, while providing abundant active sites and enhancing the rapid electrolyte ion/electron diffusion kinetics.
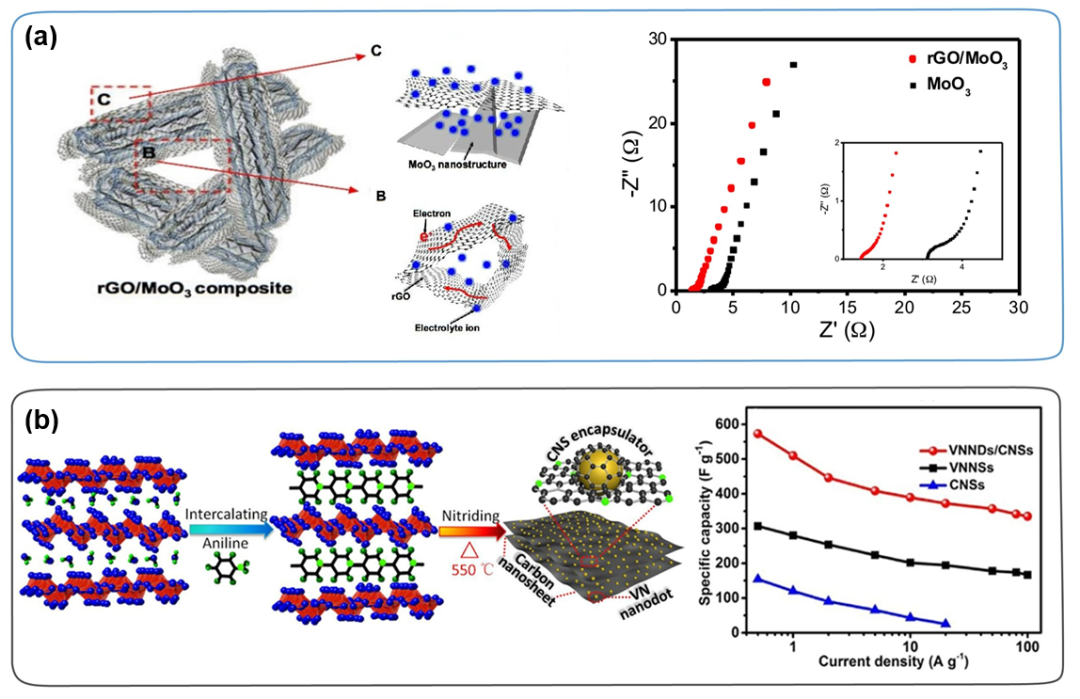
Figure 9. (a) Schematic diagram of the structure and synergistic effect of rGO/MoO₃ composite material, and the Nyquist diagram of rGO/MoO₃ composite material and MoO₃. (b) Schematic diagram of the preparation process of VNNDs/CNSs and the specific capacitances of VNNDs/CNSs, VNNSs, and CNSs at different current densities.
So far, although a lot of research has been done on the interface design of TMC/carbon, it is still a huge challenge to achieve the excellent energy density and superior power density of the electrode material, and the key challenge is to establish the relationship between TMC and carbon. Stable interface combination. Combining physical space constraints and chemical bonding may be a more effective way to achieve rapid electron transfer at the TMC/carbon-based electrode interface in the future.
III Adjustment of TMC electronic structure
For most TMCs, the regulation of electronic structure plays a vital role in improving the electrochemical performance of supercapacitors. For example, due to the different connection methods, manganese oxide exhibits a rich crystal structure (including α, β, γ, δ, and λ forms), resulting in different electronic structures. Especially when the crystal size of TMC is reduced to nanometer level or quantum dot level, due to special effects such as small size effect, quantum tunneling effect and surface effect, the physical and chemical properties of the material will change significantly. In addition, the doping of multi-phase atoms adjusts the electronic structure of the crystal, thereby generating a synergistic effect of multiple metals, and can also further improve electrochemical performance.
3.1 Nanocrystals or quantum dots
Due to slow reaction kinetics, poor conductivity, volume expansion effect, etc., it is difficult for TMC-based electrodes to achieve high power density and long cycle life in the practical application of supercapacitors. In order to solve the above-mentioned challenges, designing TMC with nanocrystalline or quantum dot size is an effective strategy. The nanostructured TMC can provide a larger electrochemically active surface due to the surface effect, shorten the ion diffusion distance, and increase the contact area with the electrolyte. For example, Wang et al. synthesized sub-nano, ultra-fine a-Fe₂O₃ flakes (SU-Fe₂O₃-rGO) supported on the surface of graphene by controlling the crystallization kinetics. Compared with NP-Fe₂O₃-rGO, SU-Fe₂O₃-rGO with ultra-thin thickness (approximately 0.9 nm) and smaller lateral size (approximately 14.5 nm) can avoid the problem of slow ion diffusion kinetics in the Fe₂O₃ bulk phase, and greatly It promotes the transport of ions/electrons in electrochemical reactions (Figure 10a-c). The results show that the sub-nano SU-Fe₂O₃-rGO exhibits the best electrochemical performance. In recent decades, research on quantum dots (QDs) in various potential applications has grown exponentially, especially in supercapacitors. For example, Xia et al. synthesized Fe₂O₃/FGS hybrid materials by thermal decomposition method, and evenly decorated Fe₂O₃ quantum dots (QDs) (about 2 nm) on functionalized graphene sheets (FGS) (Figure 10d). The results show that the asymmetric supercapacitor based on Fe₂O₃/FGS has a high energy density of 50.7 Wh kg⁻1 when the power density is 100 W kg⁻1. After 5000 cycles in the 2 V voltage window, the cycle stability reaches 95 %.
Figure 10. (a) Schematic diagram of growing SU-Fe₂O₃ flakes on graphene. (b) TEM image of the hybrid product of SU-Fe₂O₃-rGO. (c) Ragone diagram related to the energy and power density of SU-Fe₂O₃-rGO//NiCo₂O₄. (d) The schematic diagram of the Fe₂O₃/FGS composite electrode and the specific capacitance of the original FGS, Fe₂O₃ and Fe₂O₃/FGS composite electrode at different scan rates.
3.2 Heteroatom doping
Heterogeneous atom doping is another effective strategy that can fundamentally adjust the electronic structure, change the charge distribution, and further improve the reactivity and electrochemical performance of TMC/carbon-based supercapacitors. According to the type of doping, heteroatom doping can be divided into cationic doping (heterogeneous metal atoms, such as Ni and Co, Co and W, Mn and Fe, and even more metal atoms, etc.) and anion doping (such as N , P or F etc.). Due to the synergistic effect of various transition metal atoms, different transition metal composite materials have higher electrical conductivity and rich redox activity, which is beneficial to improve electrochemical performance. For example, Liu et al. systematically studied the influence of cobalt and manganese atoms (Co, Mn) on the single-doping or co-doping of Ni(OH)₂ crystals through density functional theory (DFT). The doping of Mn can increase the capacity, because the deprotonation energy of Mn is lower and the electron transfer path is easier, and the doping of Co can improve the structural stability of the entire electrode (Figure 11). As shown in Figure 11b-d, when the power density of the Ni-Co-Mn-OH/rGO//PPD/rGO hybrid capacitor is 1.68 and 18.5 kW kg⁻1, the energy density is 74.7 and 49.9 W kg⁻1, respectively. In addition, after 10,000 cycles of 20 A g⁻1, its initial capacity can maintain 91%, showing super-high energy storage capacity and long cycle life.
3.3 Defects
Defects, such as holes, edges, grain boundaries, replacement impurities, etc., have always played an important role in improving the geometric structure and electronic structure of TMC. Different defects often play different roles in improving the electrochemical performance of TMC. For example, vacancies add more active sites, improve conductivity, and substitute impurities provide additional capacitance. In particular, metal vacancies and non-metal vacancies have been extensively explored to improve the intrinsic conductivity and redox activity of TMC. For example, Liu et al. successfully prepared F-Co₂MnO₄₋ₓ/CF composite materials by introducing oxygen vacancies in Co₂MnO₄ (Figure 12a). Compared with F-Co₂MnO₄/CF (204 mAh g⁻1, 90.9%) and Co₂MnO₄/CF (165 mAh g⁻1, 85.8%), F-Co₂MnO₄₋ₓ/CF exhibits a higher specific capacity (269 mAh g) ⁻1) and better cycle stability (93.3% after 5000 cycles), which shows that the introduction of F dopants and oxygen vacancies can synergistically improve the conductivity and reactivity of electrochemically active sites. In addition, F-Co₂MnO₄₋ₓ/CF//Fe₂O₃/CF ASCs has a high energy density of 64.4 Wh kg⁻1 when the power density is 800 W kg⁻1 (Figure 12b-c).
3.4 Heterogeneous interface
In recent years, due to the higher ion carrier mobility and wider electrode potential, the construction of heterogeneous interfaces has aroused extensive research interest. This is mainly because its unique structure can reduce the activity of hydrogen evolution reaction (HER) and oxygen evolution reaction (OER) under the high voltage window. For example, using a new combination of hydrothermal reaction, phosphating treatment and electrodeposition technology strategies, NiFeP@NiCo₂S₄/carbon cloth (NiFeP@NiCo₂S₄/CC) hybrid electrode with NiFeP@NiCo₂S₄ quality structure was successfully prepared (Figure 13a). Compared with NiFeP/CC and NiCo₂S₄/CC, NiFeP@NiCo₂S₄/CC composite electrode has a higher specific capacitance (Figure 13c), better rate performance and better cycle stability. In the asymmetric supercapacitor of NiFeP@NiCo₂S₄/CC//porous carbon OPC-850, the device achieved a high energy density of 32.1 Wh kg⁻1 at an ultra-high power density of 18034.2 W kg⁻1 (Figure 15d). Its excellent electrochemical performance can be attributed to its unique heterostructure not only provides abundant open channels to achieve rapid electron transmission, but also expands the accessible two-dimensional surface, providing more activity for the adsorption of electrolyte ions Site (Figure 13b).
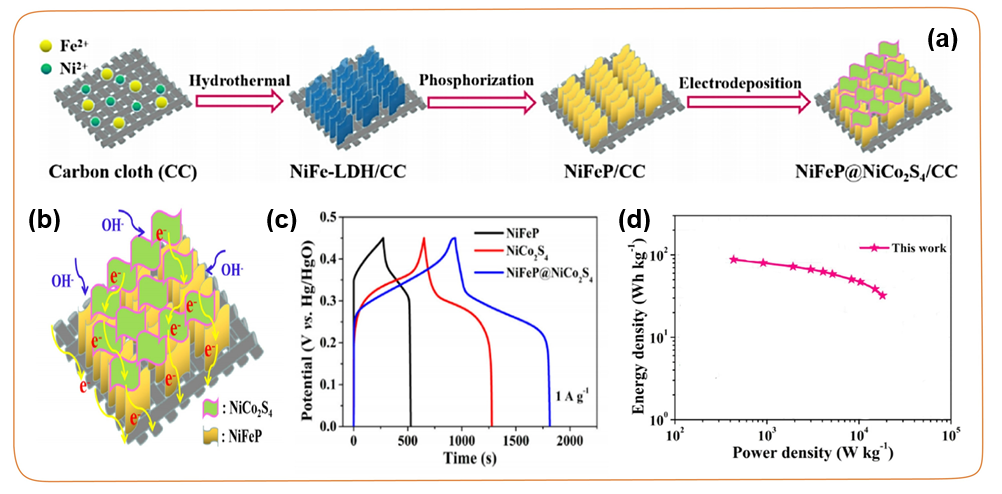
IV Conclusions and prospects
Based on the current basic understanding, challenges and opportunities, this paper systematically discusses the design strategy of TMC/carbon-based supercapacitors from the aspects of conductive carbon framework, interface engineering and electronic structure modification for the first time. Although people have done a lot of research on the assembly strategy, electrochemical performance and energy storage mechanism of TMC/carbon-based supercapacitors, some key challenges still need to be considered:
(1) How to realize the efficient use of carbon as a conductive carrier plays a very important role. Although the large specific surface area and porous structure of carbon-based materials can provide high conductivity and abundant electrolyte ion transfer channels, carbon materials with low specific capacitance limit their development. Therefore, the efficient use of carbon as a conductive substrate needs to be considered on the basis of an appropriate mass ratio between carbon and TMC, so as to achieve high energy density and high power density.
(2) The trade-off between high-quality loading and agglomeration of nanoscale TMC crystals is also a challenge. Nanostructured TMC can achieve high-quality loads and efficient utilization, with higher Faraday pseudocapacitance reaction kinetics. However, in actual situations, the ultra-small size of TMC has high surface energy and is prone to agglomeration, resulting in slow reaction kinetics like bulk materials.
(3) New technologies and methods are urgently needed to construct a stable composite interface. For composite materials, the rapid transfer of electrolyte ions/electrons on the heterogeneous interface is an important factor in achieving ultra-high charge-discharge rates. However, how to build a stable composite interface is still a problem that requires in-depth consideration.
(4) In addition, the selection of electrolyte, current collector, diaphragm and other components is also important. It is worth noting that since no additional adhesives, conductive agents or collectors are needed, the form of independent thin-film electrodes has attracted more and more attention. Compared with powder electrodes, it can increase the mass load of active materials, simplify the electrode preparation process, and avoid uncontrollable side reactions. This may be an important development direction for the energy storage industry in the future.
This article has conducted a comprehensive and in-depth discussion on the structure design strategy of high-power TMC/carbon-based supercapacitors. Trying to present classic and latest research results, and put forward an advanced perspective to solve the key challenges related to practical and large-scale applications of TMC/carbon-based supercapacitors. In general, we look forward to the emergence of more novel design strategies and high-efficiency TMC/carbon-based supercapacitors, which will further change our lifestyle in the future.
This information is sourced from the Internet for academic exchanges. If there is any infringement, please contact us to delete it immediately
18915694570
Previous: Li Yanmei/Yu Xuefeng B